Neural vs Charged Objects
If an atom contains equal numbers of protons and electrons, the atom is described as being electrically neutral. On the other hand, if an atom has an unequal number of protons and electrons, then the atom is electrically charged (and in fact, is then referred to as an ion rather than an atom). Any particle, whether an atom, molecule or ion, that contains less electrons than protons is said to be positively charged. Conversely, any particle that contains more electrons than protons is said to be negatively charged.
Electrons are migrants
The addition of energy to an atom can persuade the electrons to leave an atom. Protons do not move.
In general, for electrons to make a move from the atoms of one material to the atoms of another material, there must be an energy source, and a low-resistance pathway.
Suppose that some method is used to impart a negative charge to an object at a given location. That location would have an excess of electrons. These excess electrons will have a repulsive response to each other and would prefer more space. Since these excess electrons are present in a conductor, there is little hindrance to their ability to migrate to other parts of the object. In an effort to reduce the overall repulsive effects within the object, there is a mass migration of excess electrons throughout the entire surface of the object. Excess electrons migrate to distance themselves from their repulsive neighbors.
What happens if the conductor acquires an excess of positive charge, ie, electrons are removed from a conductor at a given location, giving the object an overall positive charge? A multitude of atoms in the region where the charging occurs have lost one or more electrons and have an excess of protons. The presence of these excess protons in a given location draws electrons from other atoms. The excess protons and both the neighboring and distant electrons attract each other. The protons cannot do anything about this attraction since they are bound within the nucleus of their own atoms. Yet, electrons are loosely bound within atoms; These electrons make the move for the excess protons, leaving their own atoms with their own excess of positive charge. This electron migration happens across the entire surface of the object, until the overall sum of repulsive effects between electrons across the whole surface of the object are minimized.
Charge as a (Scalar) Quantity
Like mass, the charge of an object is a measurable scalar quantity. The charge possessed by an object is often expressed using the scientific unit known as the Coulomb. To illustrate the magnitude of 1 Coulomb, an object would need an excess of 6.25 x 1018 electrons to have a total charge of -1 C. And of course an object with a shortage of 6.25 x 1018 electrons would have a total charge of +1 C. Because one Coulomb of charge is a large quantity of charge, the units of microCoulombs (µC) or nanoCoulombs (nC) are more commonly used as the unit of measurement of charge.
The magnitude of charge on an electron is e = 1.6 x 10-19 Coulomb
10−6 C µC microcoulomb
10−9 C nC nanocoulomb
Electric Force
The electric force is a non-contact force.
Electron Affinity
The interaction between two like-charged objects is repulsive.
The interaction between two oppositely charged objects is attractive.
Any charged object - whether positively charged or negatively charged - will have an attractive interaction with a neutral object. Positively charged objects and neutral objects attract each other; and negatively charged objects and neutral objects attract each other.
Examples of attraction between charged objects and neutral objects: 1) if a charged balloon is held above neutral bits of paper, the force of attraction for the paper bits will be strong enough to overwhelm the downward force of gravity and raise the bits of paper off the table. 2) If a charged plastic tube is held above some bits of paper, the tube will exert an attractive influence upon the paper to raise it off the table. 3) A charged rubber balloon can be attracted to a wooden cabinet with enough force that it sticks to the cabinet.
When objects repel each other, one can be certain that both objects are charged.
When objects attract each other, at least one of the objects is charged.
Conductors, Semiconductors, Superconductors, and Insulators
Conductors are materials that permit electrons to flow freely from particle to particle. An object made of a conducting material will permit charge to be transferred across the entire surface of the object. If charge is transferred to the object at a given location, that charge is quickly distributed across the entire surface of the object. The distribution of charge is the result of electron movement.
Insulators are materials that impede the free flow of electrons from atom to atom and molecule to molecule. If charge is transferred to an insulator at a given location, the excess charge will remain at the initial location of charging. The particles of the insulator do not permit the free flow of electrons; subsequently charge is seldom distributed evenly across the surface of an insulator.
Semiconductors are are between conductors and insulators. They are less conducting than most metals, but more conducting than most insulators. Examples of semiconductors are silicon and germanium.
Superconductors offer absolutely no resistance to the flow of charge. It is a perfect conductor of electric charge. Many metals and ceramics become superconducting when they are brought to extremely low temperatures.
Polarization
In the context of electricity, polarization is the process of separating opposite charges within an object. The positive charge becomes separated from the negative charge. By inducing the movement of electrons within an object, one side of the object is left with an excess of positive charge and the other side of the object is left with an excess of negative charge. Charge becomes separated into opposites.
Polarization can occur within insulators, but the process occurs in a different manner than it does within a conductor. In a conducting object, electrons are induced into movement across the surface of the conductor from one side of the object to the opposite side. In an insulator, electrons merely redistribute themselves within the atom or molecules nearest the outer surface of the object.
Polarization in conductors
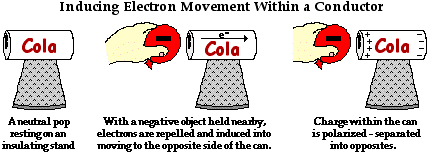
Polarization in insulators
Charging by friction
The frictional charging process results in a transfer of electrons between the two objects that are rubbed together. Rubber has a much greater attraction for electrons than animal fur. As a result, the atoms of rubber pull electrons from the atoms of animal fur, leaving both objects with an imbalance of charge. The rubber balloon has an excess of electrons and the animal fur has a shortage of electrons. Having an excess of electrons, the rubber balloon is charged negatively. Similarly, the shortage of electrons on the animal fur leaves it with a positive charge. The two objects have become charged with opposite types of charges as a result of the transfer of electrons from the least electron-loving material to the most electron-loving material.
Charging by conduction
Charging by induction
Induction charging is a method used to charge an object without actually touching the object to any other charged object.
Grounding
A discussion of charging would not be complete without a discussion of uncharging. Objects with an excess of charge - either positive or negative - can have this charge removed by a process known as grounding. Grounding is the process of removing the excess charge on an object by means of the transfer of electrons between it and another object of substantial size. When a charged object is grounded, the excess charge is balanced by the transfer of electrons between the charged object and a ground. A ground is simply a larger object (compared with the charged object) that serves as a seemingly infinite reservoir of electrons; the ground is capable of transferring electrons to or receiving electrons from a charged object in order to neutralize that object.
Electric Force and Electric Field
Electric force is an action-at-a-distance force. Action-at-a-distance forces are also called field forces. The concept of a field force is utilized by scientists to explain the force phenomenon that occurs in the absence of physical contact.
Electric field is a vector quantity whose direction is defined as below.
Electric field intensity is the strength of an electric field at any point. It is equal to the electric force per unit charge experienced by a test charge placed at that point. The unit of measurement is newtons per coulomb, or volts per meter (1 Volt is equivalent to one Joule per Coulomb, and 1 joule = 1 newton meter).
Electric Potential Energy vs Electric Potential
To move a charge in an (external) electric field against its natural direction of motion would require work. The exertion of work by an external force would in turn add potential energy to the object. The natural direction of motion of an object is from high energy to low energy, and work is not required to move an object from high energy to low energy.
Electric potential energy is dependent upon at least two types of quantities:
1) Electric charge - a property of the object experiencing the electrical field, and
2) Distance from source - the location within the electric field
While electric potential energy has a dependency upon the charge of the object experiencing the electric field, electric potential is purely location dependent. Electric potential is the potential energy per charge.
Electric Potential V = Potential Energy (joule) / Charge (coulomb)
The unit of electric potential is volt, which is Joule / Coulomb
Coulomb’s Law
The electrostatic force between two point charges, distance r apart, is directly proportional to the magnitudes of the charges and inversely proportional to the square of the distance between their centres.
Electric Force Fq = k (q1q2/r2)
k = 8.99 * 10 9 N * m2/C2
Electric Field Intensity
Electric field intensity is electric force per unit charge:
Electric field intensity E = Fq / q (where q is magnitude of the test charge)
Magnitude of elctric field intensity |E| = k (Q/r2) (where Q is magnitude of the source charge)
Electric Potential & Electric Potential Energy
Electric Potential Energy PE = Fq * r = k * q1 * q2 / r = V * q (where q is magnitude of the test charge)
Electric Potential V = k * Q / r (where Q is magnitude of the source charge)
Electric Potential Difference ΔV = ΔPE / q (where q is magnitude of the test charge)
Remember: Electric force per unit charge is electric field intensity; electric potential energy per unit charge is electric potential.
Electric Field Intensity & Potential Difference
Consider definition of work W:
W = Fq Δd = q E Δd, where E is electric field intensity
ΔPE (change in electric potential energy) = -W = - Fq Δd = - q E Δd
Therefore we have:
ΔV = ΔPE / q = - E Δd, or:
E = - ΔV / Δd
Electric Force Direction
A positive test charge moves from high potential to low potential
A negative test charge moves from low potential to high potential
In both cases, the electric potential energy decreases as it is converted to kinetic energy.
Also remember that the potential of a single point in space in an electric field is relatively meaningless. Instead you need to find the potential difference between an initial and final position. As with gravity, it is the relation to another point that matters, and moving a charge from 1 point o felectrical potential to another is what generates (kinetic) energy.
Isolines (Equipotential Lines)
Isolines (equipotential lines) are lines that connect points of equal electric potential values. As shown in the diagram below, we have an isolated point charge Q with its electric field lines in blue and isolines (equipotential lines) in green. The potential is the same along each equipotential line, meaning that no work is required to move a charge anywhere along one of those lines. Work is needed to move a charge from one equipotential line to another. Equipotential lines are perpendicular to electric field lines in every case.
Uniform Electric Field Intensity between 2 Parallel Charged Plates
- The electric field intensity is uniform at all points between the parallel plates, independent of position.
- The magnitude of the electric field intensity |E| at any point between the plates is proportional to the charge density on the plates q/A
- The electric field intensity in the region outside the plates is very low (close to zero), except for the fringe effects at the edges of the plates.
- Electric field intensity E = V / d, where V is potential difference between plates (in volts), and d is distance between plates (in metres)
- In the diagram below, the dotted lines are the isolines (equipotential lines), and the solid blue lines are the field lines. The isolines are perpendicular to the electric field lines.
Electric Potential in Circuits
A battery powered electric circuit has locations of high and low potential. The positive terminal is described as the high potential terminal, and the negative terminal is described as the low potential terminal. This assignment of high and low potential to the terminals of an electrochemical cell presumes the traditional convention that electric fields are based on the direction of movement of positive test charges.
Within the electrochemical cells of the battery, there is an electric field established between the two terminals, directed from the positive terminal towards the negative terminal. The movement of a positive test charge through the cells from the negative terminal to the positive terminal would require work, thus increasing the potential energy of every Coulomb of charge that moves along this path.
The movement of positive charge through the wires from the positive terminal to the negative terminal would occur naturally. Such a movement of a positive test charge would be in the direction of the electric field and would not require work. The charge would lose potential energy as it moves through the external circuit from the positive terminal to the negative terminal.
Electric Potential Difference (Voltage)
The difference in electric potential is represented by the symbol ΔV and is formally referred to as the electric potential difference. By definition, the electric potential difference is the difference in electric potential (V) between the final and the initial location when work is done upon a charge to change its potential energy. In equation form, the electric potential difference is
ΔV = Vb - Va = work/Charge = ΔPE/Charge
The standard metric unit on electric potential difference is the volt, abbreviated V. One Volt is equivalent to one Joule per Coulomb. If the electric potential difference between two locations is 1 volt, then one Coulomb of charge will gain 1 joule of potential energy when moved between those two locations. If the electric potential difference between two locations is 3 volts, then one coulomb of charge will gain 3 joules of potential energy when moved between those two locations. And finally, if the electric potential difference between two locations is 12 volts, then one coulomb of charge will gain 12 joules of potential energy when moved between those two locations. Because electric potential difference is expressed in units of volts, it is sometimes referred to as the voltage.
Current
Current is the rate at which charge flows past a point on a circuit. Current is a rate quantity. current as a rate quantity would be expressed mathematically as
Current = I = Q/t
The standard metric unit for current is the ampere. Ampere is often shortened to Amp and is abbreviated by the unit symbol A. A current of 1 ampere means that there is 1 coulomb of charge passing through a cross section of a wire every 1 second.
1 Amp = 1 coulomb / second
Conventional Current Direction
Conventionally, the electric field direction within a circuit is by definition the direction that positive test charges are pushed. However, the particles that carry charge through metal wires in a circuit are mobile electrons. Thus, these negatively charged electrons move in the direction opposite the electric field and the conventional current direction.
While electrons are the charge carriers in metal wires, the charge carriers in other circuits can be positive charges, negative charges or both. In fact, the charge carriers in semiconductors, street lamps and fluorescent lamps are simultaneously both positive and negative charges traveling in opposite directions.
Drift speed of electrons
Drift speed refers to the average distance traveled by a charge carrier per unit of time. The drift speed of an electron moving through a wire is very slow. The path of a typical electron through a wire could be described as a rather chaotic, zigzag path characterized by collisions with fixed atoms. Each collision results in a change in direction of the electron. And because of collisions with atoms in the solid network of the metal conductor, there are two steps backwards for every three steps forward. With an electric potential established across the two ends of the circuit, the electron continues to migrate forward. Progress is always made towards the positive terminal. Yet the overall effect of the countless collisions and the high between-collision speeds is that the overall drift speed of an electron in a circuit is abnormally low. A typical drift speed might be 1 meter per hour.
How can there be a current on the order of 1 or 2 ampere in a circuit if the drift speed is only about 1 meter per hour? The answer is: there are many, many charge carriers moving at once throughout the whole length of the circuit.
Another question: if the average drift speed of an electron is very, very slow, why does the light in a room or in a flashlight light immediately after the switched is turned on? Once the switch is turned to on, the circuit is closed and an electric potential difference is established across the two ends of the external circuit. The electric field signal travels at nearly the speed of light to all mobile electrons within the circuit, ordering them to begin marching. As the signal is received, the electrons begin moving along a zigzag path in their usual direction. Thus, the flipping of the switch causes an immediate response throughout every part of the circuit, setting charge carriers everywhere in motion in the same net direction. While the actual motion of charge carriers occurs with a slow speed, the signal that informs them to start moving travels at a fraction of the speed of light.
Power
In a circuit, a light bulb, beeper and motor are generally referred to as a load. In a light bulb, electrical energy is transformed into useful light energy (and some non-useful thermal energy). In a beeper, electrical energy is transformed into sound energy. And in a motor, electrical energy is transformed into mechanical energy.
Power is the rate at which electrical energy is supplied to a circuit or consumed by a load. Power is the rate at which work is done. Like current, power is a rate quantity. Its mathematical formula is expressed on a per time basis:
Power = Work done on charge / time = Energy consumed by load / time.
The unit of electrical power is the watt, abbreviated W. A watt of power is equivalent to the delivery of 1 joule of energy every second. In other words: 1 watt = 1 joule / second
If a light bulb is rated at 60 watts, then there are 60 joules of energy delivered to the light bulb every second. A 120-watt light bulbs draws 120 joules of energy every second.
Electrical utility bills usually have a charge for the number of kilowatt-hours of electricity that were consumed. Exactly what is a kilowatt-hour? A kilowatt is a unit of power and an hour is a unit of time. So a kilowatt • hour is a unit of Power • time. If Power = ΔEnergy / time, then Power • time = ΔEnergy. So a unit of power • time is a unit of energy. The kilowatt • hour is a unit of energy.
Resistance
Resistance is the hindrance to the flow of charge.
- First, the total length of the wires will affect the amount of resistance. The longer the wire, the more resistance that there will be.
- Second, the cross-sectional area of the wires will affect the amount of resistance. When all other variables are the same, charge will flow at higher rates through wider wires with greater cross-sectional areas than through thinner wires.
- A third variable that is known to affect the resistance to charge flow is the material that a wire is made of. Not all materials are created equal in terms of their conductive ability. Some materials are better conductors than others and offer less resistance to the flow of charge.
R = pL/A, where R is resistence (unit is ohm), L is length in meters, A is cross-sectional area in meter2, and p is the material's resistivity, usually measured at 20 degrees Celsius.
Ohm's Law
ΔV = I • R
I = ΔV/R
Some derived equations for power
P = ΔV • I
P = I2 • R
P = ΔV2 / R
Series Circuits
Req = R1 + R2 + R3 + ...
Ibattery = I1 = I2 = I3 = ...=ΔVbattery / Req
ΔVbattery = ΔV1 + ΔV2 + ΔV3 + ...
Parallel Circuits
Itotal = I1 + I2 + I3 + ...
1 / Req = 1 / R1 + 1 / R2 + 1 / R3 + ...
ΔVbattery = ΔV1 = ΔV2 = ΔV3 = ...
Electrical Schematic Diagram
Ammeter and Voltmeter
Ammeter measures the current at a point in the circuit, voltmeter measures potential difference between 2 points in the circuit. Ammeter is placed in series with the load, voltmeter is placed in parallel with the load.
Capacitors
What is capacitor and how does it work?
https://m.youtube.com/watch?v=L6cgSxpGmDo
Capacitor use - Decoupling (Bypass) Capacitors
Capacitor use - AC to DC Converter
What is capacitance?
The capacitance C of a capacitor is defined as the charge Q of the capcitor divided by the potential difference V on the capcitor to bring its charge to Q: C = Q / V. The SI unit of capacitance C is Farad (F).
The capacitance of a given capacitor is a property of the materials used and the geometry of the 2 surfaces. For simple parallel plate capacitors, it can be shown that C = Q / V = ε0A/d, where A is the cross-sectional area of the plates, and d is the distance between them.
The energy stored in a capacitor can be studied by recognizing that given C=Q / V, we have Q = C * V. Thus we have a linear relationship between plate charge and voltage. If we plot a graph of charge versus voltage, we will have the following straight line, and the area below the diagonal straight line will be the amount of energy stored in the capacitor.
Energy stored in a capacitor PE = (1/2) QV = (1/2) CV2 = (1/2) Q2/C
Dielectric
Dielectric is an insulating material that is used to seperate the 2 metal plates of a capacitor. A dielectric will always increase the capacitance of a capacitor:
Cwith dielectric = kCwithout dielectric, where k is the dielectric constant, which varies from material to material and is always >1.
Capacitors in series circuits
V1 + V2 = V
Q/C1 + Q/C2 = Q/C
1/C1 + 1/C2 = 1/C
Capacitors in parallel circuits
Q = Q1 + Q2
CV = C1V + C2V
C = C1 + C2
Diodes
Practice Questions
Question 1
Find the electric field created by the charges A and B at point C in terms of k.q/d2?
Question 2
If the electric field at point A is zero, find the charge at point D in terms of q.
Question 3
Find the change in electric potential energy, , as a charge of (a)
or (b)
moves from a point A to a point B, given that the change in electric potential between these points is
.
Solution:
(a)
(b)
Question 4
A uniform electric field is established by connecting the plates of a parallel-plate capacitor to a 12 V battery. (a) If the plates are separated by 0.75 cm, what is the magnitude of the electric field in the capacitor? (b) A charge of moves from the positive plate to the negative plate. Find the change in electric potential energy for this charge. (In elecrical systems we shall assume that gravity can be ignored, unless specifically instructed otherwise.)
Solution:
(a) Using we solve for E and substitute the values:
(b) Using :
Question 5
The electric potential at point B in the parallel-plate capacitor shown below is less than the electric potential at point A by 4.50 V. The separation between points A and B is 0.120 cm, and the separation between the plates is 2.55 cm. Find (a) the electric field within the capacitor and (b) the potential difference between the plates.
Solution:
(a) Using
(b) Using
Question 6
In a certain region of space the electric potential V is known to be constant. Is the electric field in this region (a) positive, (b) zero, or (c) negative?
Solution:
(b) The electric field is zero.